All-optical electrophysiology initiates and tracks electrical activity in excitable cells such as neurons and cardiomyocytes using optically-actuated depolarization coupled with voltage-sensitive fluorescence detection. It is implemented using optogenetic technology in tandem with imaging of voltage-sensitive dyes (VSD) or genetically encoded voltage indicators (GEVI) [1]. Although microelectrode-based electrophysiology remains unsurpassed in its ability to quantify small currents and membrane potential changes in single neurons, all-optical electrophysiology is better suited to interrogating the spatial complexity of neuronal networks and identifying functional phenotypes associated with disease states [2].
Optogenetics and GEVIs are relatively recent developments. In contrast, VSDs have a history of gradual improvement extending over more than 50 years. VSD imaging is inherently challenging, requiring detection of small changes in small fluorescence signals. A functional VSD must be confined to the cell membrane, which constitutes about 0.1% of the total cell volume. Voltage- sensitive fluorescence signals result from the Stark effect — modulation of the dye’s electronic configuration by the transmembrane electric field. The extent of modulation is weak, such that fluorescence changes are typically ~10% per 100 mV. Consequently, accurate measurements require very stable excitation light. LED sources excel in this respect [3], as do other solid-state lighting technologies. Fluorescent protein-based GEVIs generally produce smaller and slower voltage- dependent fluorescence changes than VSDs [4]. Their primary advantage is the capacity for selective labeling of specific cell types. This capacity is particularly important in intact tissues where adjacent cells may perform distinct functions. Furthermore, GEVIs and optogenetic actuators can be encoded in a single vector and co-expressed [1].
Illumination sources for all-optical electrophysiology applications must be capable of delivering two or more spectral outputs with millisecond temporal control. Solid-state Light Engines with either LED or laser sources meet these requirements. In general, lasers provide higher irradiance in smaller areas than LEDs. Lasers are preferred to LEDs for in vivo optogenetics applications due to their superior coupling efficiency into 200 μm diameter multimode optical fibers. Spectral outputs for stimulation and VSD response detection must be spectrally well separated so that the relationship between stimulus and response is not distorted by optical crosstalk. A typical configuration is shown in Figure 1. Depolarization is initiated by a brief pulse (5-50 ms) of light, spectrally tuned to the absorbance or action spectrum of the optogenetic actuator channelrhodopsin (ChR2). The VSD (BeRST 1) is excited with red light, generating voltage-sensitive fluorescence responses of 24% per 100 mV. Following ChR2 stimulation (475 nm, 0.8 mW/mm2, SPECTRA X Light Engine, Lumencor, Inc., Beaverton, Oregon), simultaneous electrophysiological and optical recordings of membrane potential changes are effectively identical [4]. In some cases, optogenetic actuation in a different wavelength range may be required. For example, light absorption by hemoglobin can inhibit photoactivation of channelrhodopsin at ~470 nm (Figure 1) in tissues, providing motivation for the development of spectrally red-shifted variants of channelrhodopsin [5]. Irradiance requirements for activation of channelrhodopsin in cultured neurons are relatively modest (1–5 mW/mm2,) but may be orders of magnitude higher in tissues where incident light is dissipated by scattering. The electrical activity of neurons is modulated on the millisecond timescale. Therefore, illumination sources used for optogenetic stimulation must have the capacity for optical modulation on the same timescale. The capacity for independent control of light intensity and timing is particularly useful for controlling the extent of ChR2-induced depolarization (Figure 2).
While electrophysiology remains a method of choice for small current and membrane potential detection in single neurons, the inherent challenges of electronic transduction relegate it to a somewhat limited scope of execution. Confounding electronic noise, challenging reproducibility, and painstaking implementation are all commonplace in standard electrophysiology techniques driven by electronic transduction. All-optical electrophysiology obviates such challenges with the use of optically actuated depolarization and voltage sensitive fluorescence for detection. Successful implementation of all optical transduction necessitates well-behaved illumination. Light sources must be bright, stable, reproducible, spectrally discrete, and ideally linearly dependent on drive current. Optical modulation must be robust on the millisecond timescale. The availability of high performance solid-state light sources in turn-key Light Engines are truly enabling in such demanding applications. Such lighting tools play an important role in the proliferation of such informative, bio-photonic cellular analyses.
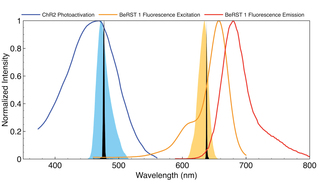
Figure 1. Output spectra of 472 nm (blue fill) and 635 nm (orange fill) LEDs and 477 nm and 639 nm diode lasers (black fills) superimposed on photoactivation spectrum of ChR2 and fluorescence excitation spectrum of VSD BeRST 1.
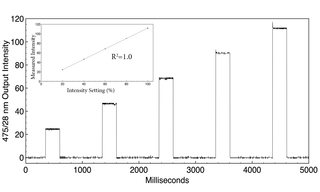
Figure 2. Filtered (475+/-14 nm) LED output from a SPECTRA Light Engine (Lumencor, Inc., Beaverton, Oregon) detected by a photodiode. Output intensity is increased by 20% for each successive 250 ms pulse. The control response is precisely linear (inset).
- Feb 13, 2023
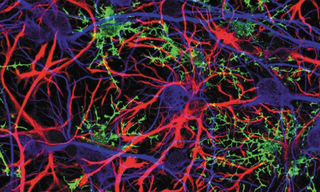
- D Wagenaar (2012) An optically stabilized fast-switching light emitting diode as a light source for functional neuroimaging. PLoS One 7:e29822(opens in new window)
- DR Hochbaum, Y Zhao, AE Cohen et al. (2014) All-optical electrophysiology in mammalian neurons using engineered microbial rhodopsins. Nat Methods 11:825–833(opens in new window)
- H Zhang, AE Cohen (2017) Optogenetic approaches to drug discovery in neuroscience and beyond. Trends Biotechnol 35:625–639(opens in new window)
- JY Lin, PM Knutsen, RY Tsien et al. (2013) ReaChR: a red-shifted variant of channelrhodopsin enables deep transcranial optogenetic excitation. Nat Neurosci 16:1499–1508(opens in new window)
- YL Huang, AS Walker, EW Miller (2015) A photostable silicon rhodamine platform for optical voltage sensing. J Am Chem Soc 137:10767–10776(opens in new window)